SaferHeart
Many factors can lead to impaired heart function ranging from malnutrition in countries where the access to high energy food is almost limitless up to the side effects of many drugs which are used in the pharmaceutical industry. Especially for cancer drugs, impairment of normal heart function has been widely reported. In this section we give insight into the mechanisms which lead to impaired heart function and introduce our approaches which we take to design and develop safer and more sustainable products.
- About
- About
- Our solutions for you
- Our services
- Request further information
About
The heart is a muscle which has the size of a closed fist and which sits in the middle of the thorax between the lungs. The heart of an adult human male weighs approximately 280 g and pumps around 5 L blood per minute. The heart consists of striated cardiac muscles which makes up the middle layer of the heart. The muscle consists of the myocardium which is surrounded by a thin epicardium and an inner endocardium.1 The muscle is covered by coronary arteries which supply the heart with oxygen containing blood and cardiac veins to transport away CO2-containing blood. The muscle is innervated by cardiac nerves which supply the electric action potentials which make the muscle contract approximately 60 times per minute in resting period through excitation-contraction coupling.
CO2 containing and O2 poor blood enters the heart from the body by the Vena cava (inferior and superior) to enter the right atrium. Through the tricuspid valve this blood enters the right ventricle from where it gets pumped to the lungs over the pulmonary valve and the pulmonary artery. In the lungs the blood exchanges CO2 by O2 and oxygen rich, CO2 poor blood is directed back to the heart over the pulmonary vein to the left atrium of the heart. By passing the mitral valve the blood enters the left ventricle from where it gets pumped back into the body circulation over the aortic valve into the aorta. The valves ensure that there is no backflow of blood into the previous compartments to keep the blood flowing into a uniform direction. An illustration of the blood flow is shown in Figure 1.
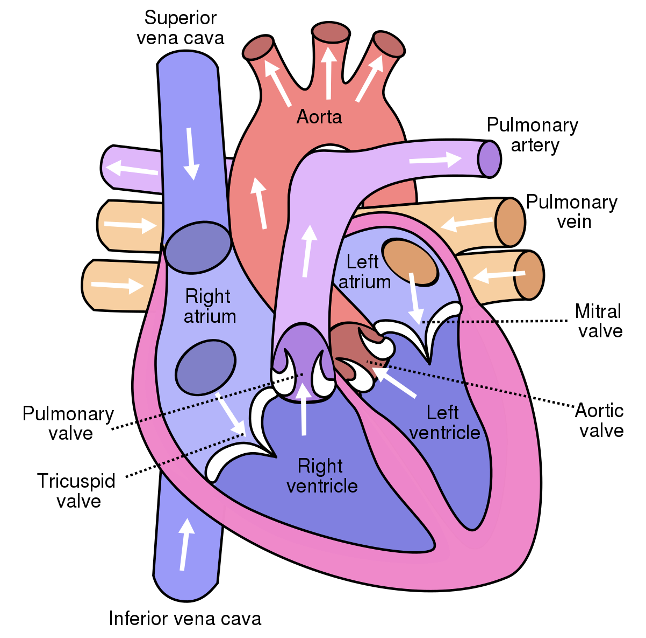
Figure 1: Scalable Vector Graphics (SVG) illustration of the human heart.2
To be able to maintain the described blood flow and to keep it functional, the muscle contractions (systoles) and relaxations (diastoles) between the atria and ventricles have to be well coordinated. If this coordinated muscle contraction gets misbalanced, heart arrhythmia can result which can lead to severe symptoms and even to death by heart failure. To coordinate muscle contraction, the action potentials which give rise to this contraction have to be well coordinated as well. The initiating electric action potential thereby is generated by the sinus node which sits on top of the right atrium and which initiates the contraction of muscles of the left and right atria. The sinus node is coupled to intrinsic oscillators and to inputs from the sympathetic and parasympathetic vagus nerve which regulate and coordinate the spontaneously generated action potentials. When measuring the contraction of the atria by an Electrocardiogram (ECG), this contraction can be seen as the P wave as illustrated in Figure 2. The action potential then reaches the atrioventricular node which delays the action potential slightly to give the atria enough time to contract. The action potential then continues along the his-bundle of the septum to reach the Purkinje fibers from where it initiates synchronised contraction of the ventricles. In the ECG, this contraction generates the QRS signal. During the ST phase of the ECG, the ventricles depolarize again to be ready for the next action potential. Having this ECG curve, we can also read the impulse conduction from the atria to the ventricles as the PQ segment and the heart rate and its regulation by investigating the PP intervals.1
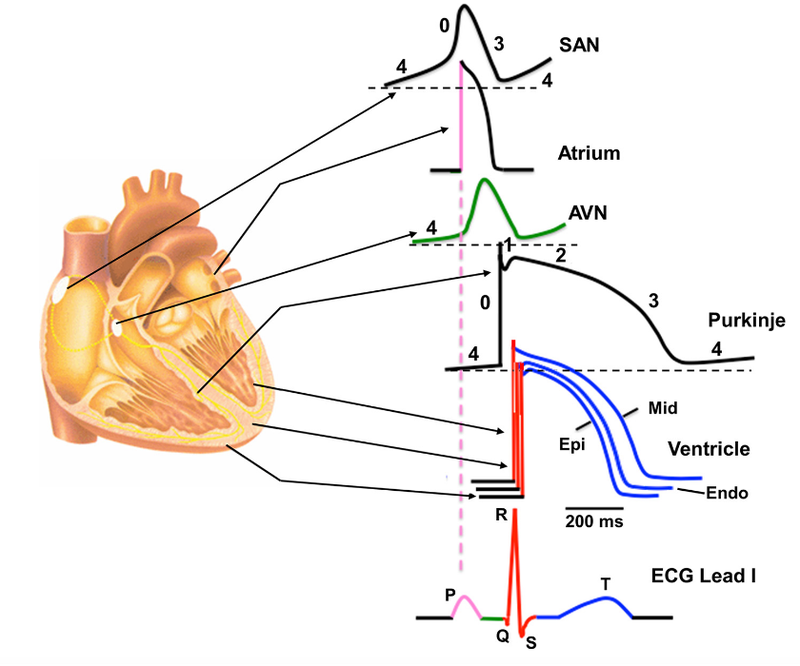
Figure 2: Regional differences in cardiac action potential configurations. SAN = Sinoatrial node, AVN = Atrioventricular node.3
The Resting Membrane Potential (RMP) of myocytes ranges from -95 to -40 mV depending on the type and location of that cell within the heart. In five phases, action potentials are initiated and prolonged throughout the myocardium. In phase 0, voltage gated sodium channels open causing a fast influx of sodium and thereby raising the membrane potential up to ranges from +30 to +40 mV. Repolarisation includes phases 1, 2 and 3. Phase 1 is characterised by decreased sodium influx, increased potassium efflux and increased chloride influx to bring the membrane potential back to negative values. The potassium efflux and chloride influx remains almost constant in phase 2 during a plateau phase. Increased potassium efflux and decreased chloride and sodium influx constitute phase 3 where large repolarization occurs towards diastolic RMP. Phase 4 represents the RMP in diastole.3
Miscoordination of the action potentials can lead to severe cardiac arrhythmia such as tachycardia and bradycardia and even result in cardiac failure leading to death. Miscoordination can be physically caused by damaged myocardium for instance where correct signal transduction might be impaired or could be caused by factors such as genetic alterations which might lead to expression alterations of ion channel related proteins, leading to impaired electric signalling. Also substances which act as cardiotoxins might cause cardiac dysfunction. Especially during cancer treatments, many drugs have been identified as cardiotoxins.4 To ensure consumer and patient safety and to design safer and more sustainable products, it is an importantaim to be able to identify potential cardiotoxins in early stages of product design. To do so, we first have to get an insight into the mechanisms of cardiotoxicity.
Known cardiotoxins impair the heart function either by damaging the heart muscle making it harder for the heart to pump blood (cardiomyopathy) or by inducing heart arrhythmias such as bradycardia and tachycardia (such as ventricular tachycardia, VT), prolonged corrected QT intervals (QTc) and Atrial Fibrillation (AF). Cardiotoxicity is well described especially for cancer drugs, which are known to impair heart function.4 Since cancer patients rely on anti-cancer medications, cardiotoxicity and its mitigation can be studied with this group.
Cardiomyopathy is separated into three main categorization types4: Type 1 cardiomyopathy which is characterized by a direct cardiomyocyte damage such as induced imbalance of the metabolite household induced by a drug, Type 2 cardiomyopathy which is characterised by indirect effects over alterations in perfusions, innervation or hormonal milieu and Type 3 cardiomyopathy which is characterised by inflammatory cell infiltration into the myocardium.
Common cardiotoxins for type 1 cardiomyopathy in conventional chemotherapies include anthracyclines, which are widely used as anti-cancer drugs. The toxic effect results from anthracyclines intercalating between DNA and RNA strands to inhibit their synthesis, iron-mediated oxidative stress and histone modifications to cause altered mitochondrial function what leads to altered electrocardiograms, arrhythmias, heart block and even cardiac arrest accompanied by pain and shortness of breath. Examples for targeted cancer therapy include trastuzumab, an antibody against HER2, which causes declines in cardiac functions and Heart Failure (HF), or the Tyrosine Kinase Inhibitors (TKIs) afatinib and osimertinib which are associated with high risk for cardiotoxicity.
To prevent toxic effects, infusion rates for medication are prolonged to reduce peak circulating concentrations, liposomal formulations are used to reduce myocardial accumulation, dexrazoxane is used as a cardioprotective agent and third-generation beta-blockers can be used to prevent the effect against anthracycline-related cardiomyopathies.
Cardiotoxins for type 2 cardiomyopathy for conventional chemotherapies include the anti-cancer drug 5-fluorouracil (5-FU). 5-Fu catabolizes to fluoroacetate which interferes with the Krebs cycle activity thereby impairing cardiac function. For targeted cancer therapy Vascular Endothelial Growth Factor (VGEF) inhibitors such as bevacizumab are reported to induce cardiotoxicity. For immunotherapies the Chimeric Antigen Receptor (CAR) T cell therapy is known to induce cardiotoxicity, since it cross recognizes titin, which is a striated muscle specific protein and hence caused death for two patients who received this medication due to Heart Failure (HF) within a few days after medication, although no preclinical toxicity had been observed for this therapy before. Also radiation in the form of radiation therapy can damage cells due to the release of oxidative stress mechanisms and metabolic abnormalities which come along with exposure to radiation.
Preventive treatment approaches include varying the mode of administration of the cancer therapeutic. Especially for radiation therapy, the reduction of dose exposure is the best approach to avoid cardiotoxicity. Also potent vasodilatory therapies can be approached which use calcium channel blockers such as diltiazem or long acting nifedipine.
A common cardiotoxin for type 3 cardiomyopathy for conventional chemotherapies is cyclophosphamide, which induces myocarditis. For targeted cancer treatment the Tyrosine Kinase Inhibitor (TKI) sorafenib is known to potentially induce cardiogenic shock with fatal outcome. Immunotherapies inhibitors for the Cytotoxic T lymphocyte antigen 4 (CTLA4) and the Programmed Cell Death 1 (PD1) ligand are known to induce cardiotoxicity. To prevent type 3 cardiotoxicity one effective way is to observe clinical symptoms throughout the therapy by the use of an electrocardiograph (ECG) for instance.
Arrhythmias
Heart arrhythmia describes the altered heart beat rhythm and includes bradycardia (slowing down the normal heartbeat) and tachycardia (accelerating the normal heartbeat).
Bradycardia
Known cardiotoxic anticancer drugs which induce bradycardia during conventional chemotherapy include paclitaxel (Kolliphor EL, formerly known as Cremophor EL), and thalidomide. For targeted cancer therapies Tyrosine Kinase Inhibitors, Vascular Endothelial Growth Factor inhibitors such as pazopanib, bevacizumab in combination with vorinostat and sunitinib, crizotinib and certinib (two ALK inhibitors) are known. Also immunotherapies can lead to bradycardia through atrioventricular blocking as a secondary mechanism to inflammatory infiltration of the myocardium and radiation therapies are known to injure the heart and induce fibrosis that lead to bradycardia.
To avoid bradycardic side effects of such treatment the only effective approach is to monitor patients and to measure their electrolyte serum levels, renal and thyroid functions. If side effects are detected, medications are to be stopped until bradycardic resolution. dromotropic and chronotropic medications such as Beta blockers, digoxin and calcium channel blockers or antiarrhythmic agents might resolve the arrhythmia.
Corrected QT (QTc) prolongation and Ventricular Tachycardia (VT)
Drugs inducing QTc and VT increase the sodium inward current and decrease the potassium outward current which leads to a prolongation of the ventricular action potential and its repolarisation period mostly by blocking voltage gated potassium channels of the H member 2 subfamily, known as the hERG channel which is encoded by the KCNH2 gene.5
Known drugs which induce QTc and VT during conventional chemotherapies include Arsenic trioxide, oxaliplatin (increasing the sodium inward current), docetaxel and 5-FU. For targeted cancer therapies Tyrosine Kinase Inhibitors (TKIs) such as dasatinib, nilotinib, pazopanib, sunitinib and vandetanib, ibrutinib, HDAC inhibitors such as romidepsin, dacinostat, vorinostat (probably by blockage of hERG channel) and inhibitors of the cyclin dependent kinases such as Ribociclib (should not be combined with tamoxifen due to increased QTc risk) are known. Again, immunotherapies, leading to inflammatory infiltration of the myocardium and radiation therapies can induce QTc and VT.
Common prevention and therapy include the observation and monitoring of patients during cancer treatment such as electrolyte serum level measurements and ECG monitoring. If arrhythmia occur, patients should be treated according to clinical guidelines.6
Atrial fibrillation
Atrial Fibrillation (AF) is special in the sense that it is not only induced by cancer treatments but also by the cancer tissue itself and that there is a correlation between the two. The risk of developing cancer/AF is significantly increased when already having AF/cancer. The exact mechanism is not fully understood but it is assumed that AF unmasks malignancies by the induction of bleeding events in tumors. Shared risk factors in AF and cancer include obesity and inflammation. In conventional chemotherapy melphalan and paclitaxel are known to be able to induce AF. For targeted cancer therapy the TKI ibrutinib is known to induce AF. In immunotherapy high levels of C-reactive protein (CRP) in the plasma, CAR T cell therapy and ICI therapy can induce AF. Radiation therapy can induce AF by the development of restrictive cardiomyopathy through radiation.
Beside the mentioned impairments, the described cardiotoxins might further lead to atrial vascular disease7, venous thromboembolism8, pulmonary hypertension9, systemic hypertension10, pericardial disease11 and valvular heart disease12. A summary of the toxins and their cardiovascular toxic effects are summarised in Figure 3.
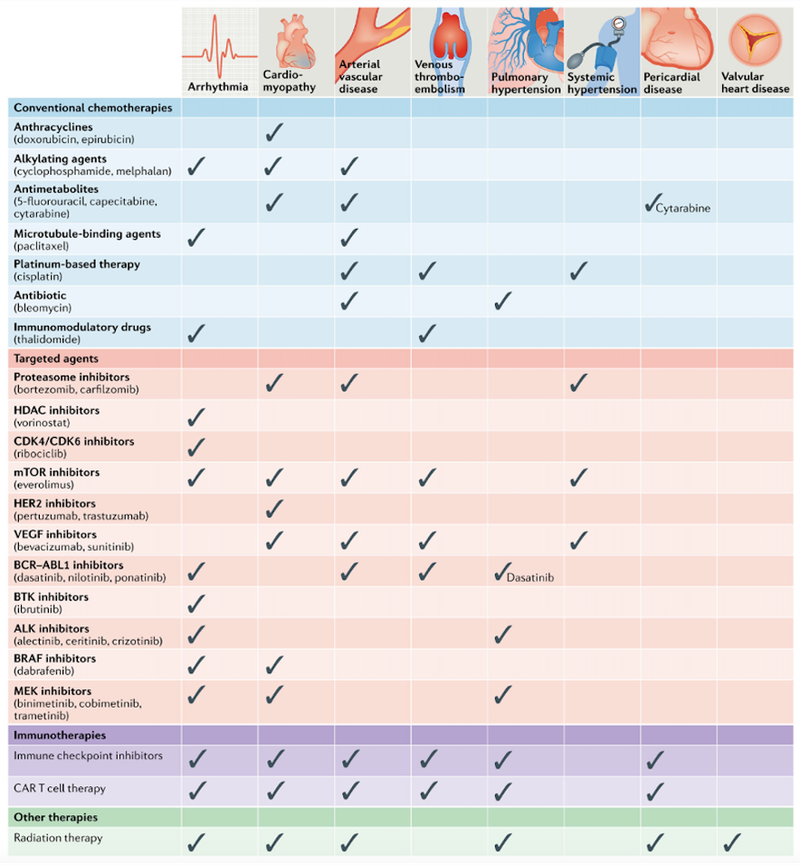
Figure 3: Outline of cardiovascular toxic effects associated with cancer therapies.4
At SaferWorldbyDesign our mission is to develop solutions with which we can identify cardiotoxins in the early stage of product design, whether it is for drug development in the pharmaceutical industry, for ingredient formulations in the food sector or any other institution which is related to the development of safe and sustainable products with concern for cardiac health. Adhering to Green Goals and the 3Rs principles, we develop our solutions based on new approach methods in in silico modeling, data management and next generation in vitro assays. Furthermore, we promote a healthy lifestyle to support the decrease in heart risks, especially of balanced nutrition plans and sport activities. With this, we aim to contribute to a safer and more sustainable world for all of us.
References
(1) Saxton, A.; Tariq, M. A.; Bordoni, B. Anatomy, Thorax, Cardiac Muscle. In StatPearls; StatPearls Publishing: Treasure Island (FL), 2023.
(2) SVG illustration of the human heart created by Wapcaplet in Sodipodi. Slightly modified for correct rendering by Yaddah (no changes to content). Cropped version without white space available at File:Diagram of the human heart (cropped).svg Uploaded to Wikimedia commons on 24 Dec 2003.
(3) Varró, A.; Tomek, J.; Nagy, N.; Virág, L.; Passini, E.; Rodriguez, B.; Baczkó, I. Cardiac Transmembrane Ion Channels and Action Potentials: Cellular Physiology and Arrhythmogenic Behavior. Physiological Reviews 2021, 101 (3), 1083–1176. https://doi.org/10.1152/physrev.00024.2019.
(4) Herrmann, J. Adverse Cardiac Effects of Cancer Therapies: Cardiotoxicity and Arrhythmia. Nat Rev Cardiol 2020, 17 (8), 474–502. https://doi.org/10.1038/s41569-020-0348-1.
(5) Slavov, S.; Zhao, J.; Huang, R.; Xia, M.; Beger, R. Prediction and Experimental Evaluation of the HERG Blocking Potential of Drugs Showing Clinical Signs of Cardiotoxicity. LDDD 2023, 20 (11), 1757–1767. https://doi.org/10.2174/1570180819666220804110706.
(6) Al-Khatib, S. M.; Stevenson, W. G.; Ackerman, M. J.; Bryant, W. J.; Callans, D. J.; Curtis, A. B.; Deal, B. J.; Dickfeld, T.; Field, M. E.; Fonarow, G. C.; Gillis, A. M.; Granger, C. B.; Hammill, S. C.; Hlatky, M. A.; Joglar, J. A.; Kay, G. N.; Matlock, D. D.; Myerburg, R. J.; Page, R. L. 2017 AHA/ACC/HRS Guideline for Management of Patients With Ventricular Arrhythmias and the Prevention of Sudden Cardiac Death: Executive Summary: A Report of the American College of Cardiology/American Heart Association Task Force on Clinical Practice Guidelines and the Heart Rhythm Society. Circulation 2018, 138 (13). https://doi.org/10.1161/CIR.0000000000000548.
(7) McCullough, P. A.; Lavie, C. J. Coronary Artery Plaque and Cardiotoxicity as a Result of Extreme Endurance Exercise. Mo Med 2014, 111 (2), 95–98.
(8) Daguenet, E.; Maison, M.; Tinquaut, F.; Giroux, E.; Bertoletti, L.; Suchaud, J.; Rancoule, C.; Guy, J.; Magné, N. Venous Thromboembolism and Radiation Therapy: The Final radiation‐induced Thrombosis Study Analysis. Cancer Medicine 2022, 11 (8), 1753–1762. https://doi.org/10.1002/cam4.4559.
(9) Gürdoğan, M. Cancer Therapy-Related Pulmonary Hypertension: A Review of Mechanisms and Implications for Clinical Practice. Anatol J Cardiol 2023, 299–307. https://doi.org/10.14744/AnatolJCardiol.2023.3013.
(10) Chung, R.; Tyebally, S.; Chen, D.; Kapil, V.; Walker, J. M.; Addison, D.; Ismail-Khan, R.; Guha, A.; Ghosh, A. K. Hypertensive Cardiotoxicity in Cancer Treatment—Systematic Analysis of Adjunct, Conventional Chemotherapy, and Novel Therapies—Epidemiology, Incidence, and Pathophysiology. JCM 2020, 9 (10), 3346. https://doi.org/10.3390/jcm9103346.
(11) Chahine, J.; Collier, P.; Maroo, A.; Tang, W. H. W.; Klein, A. L. Myocardial and Pericardial Toxicity Associated With Immune Checkpoint Inhibitors in Cancer Patients. JACC: Case Reports 2020, 2 (2), 191–199. https://doi.org/10.1016/j.jaccas.2019.11.080.
(12) Zahler, D.; Arnold, J. H.; Bar-On, T.; Raphael, A.; Khoury, S.; Rozenbaum, Z.; Banai, S.; Arbel, Y.; Topilsky, Y.; Laufer-Perl, M. Valvular Heart Disease Following Anthracycline Therapy—Is It Time to Look beyond Ejection Fraction? Life 2022, 12 (8), 1275. https://doi.org/10.3390/life12081275.
Our services
An example - The hERG channel
One of the solutions which we approach at SaferWorldbyDesign is focused on the voltage gated potassium channels of the H member 2 subfamily, known as the hERG channels.
The abbreviation hERG stands for human Ether-a-go–go Related Gene (hERG) which encodes the pore forming subunit of a delayed rectifier voltage gated K+ (VGK) channel in Drosophila.1 In humans there exist an ortholog protein which is encoded by the KCNH2 gene on chromosome 7 and which is expressed in the heart, various brain regions, smooth muscle cells, endocrine cells and a wide range of tumor cell lines. Mutations in these genes are related to chromosome 7-associated long QT syndrome (LQST). It could be shown that those channels are also targeted by class 3 antiarrhythmic drugs and that they are even involved in the development of severe diseases such as schizophrenia.1
In healthy individuals, the inactivated hERG channels are slowly activated during depolarization. After repolarization, the channels are rapidly inactivated in a voltage dependent mechanism. With this, hERG channels constitute a major factor to regulate cellular action potentials and the QT intervals.2 This channel functionality has been shown to be impaired by many currently used pharmacophores such as anti cancer reagents.4 To develop pharmacophores which do not impair hERG function, it is valuable to develop fast and efficient models with which cardiotoxins can be identified in the early stages of product design.
There have been first approaches to develop predicting non clinical models which are based on the International Conference on Harmonisation of Technical Requirements for Registration of Pharmaceuticals for Human Use (ICH) Harmonised Tripartite Guideline S7B on The Non-Clinical Evaluation of the potential for delayed ventricular repolarization (QT interval prolongation) by human pharmaceuticals from 12th May 2005.3 The guidelines are based on the concept which is illustrated in Figure 1.3
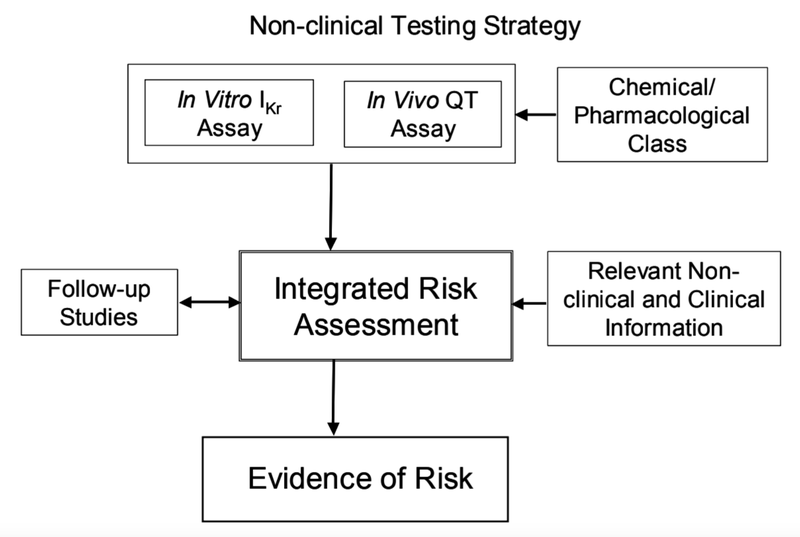
Figure 1: Non clinical testing strategy.3
Beside the in vitro procedure proposed by that guideline, in silico models might serve as a first tier where chemicals and materials can be assessed in a faster and more sustainable way.
Indeed, there have been in silico approaches which are based on Quantitative Structure-Activity Relationships (QSAR) and classification models.1 Those models correlate toxic adverse effects with the molecular structure of known compounds. With this, toxic effects can be predicted for new compounds.
It has been shown that compounds which impair hERG function share specific molecular features based on which in silico models can be developed. Those structural features include:1,2
- A basic amine core attached by a short linker to a phenyl ring.
- A para-substituent which is attached to the phenyl ring in addition to two lipophilic substituents of the amine (one or more of which may be aromatic)
Interestingly, those features are also found in the chemical structure of phospholipidosis (PLD) inducers.2 Indeed, there have been correlations found between the two and it could be shown that the distance between the centroids of the two aromatic rings plays a key element in determining whether a compound impairs hERG function or induces PLD. If the distance between the centroids is about 5.5Å, then a PLD inducer would also block the hERG channel. If the centroids are further apart than 5.5Å, the compound would no longer be a PLD inducer and remain with blocking hERG.
Modelling
Since structure is a key element for the prediction of toxic adverse effects of a compound, one first step in hERG modelling is the determination of the 3D structures of a specific test compound which then can be used for predictions.
Our services
At SaferWorldbyDesign we offer integrated services which combine the mentioned hERG modelling approach with an efficient data management infrastructure to be able to use the needed data in an efficient and straightforward way. We also participate in topic related international research projects to constantly elaborate new solutions and thereby improve the current ones. With our strong partner consortium we combine our methods with a wide variety of further services in a tiered approach including next generation in vitro assays as well as further data management and in silico modelling solutions.
With this we are able to adapt our solutions to your specific needs and interests in all facets of cardiotoxicity. If you want us to help you to develop a solution for you, please feel free to contact us!
References
(1) Vandenberg, J. I.; Perry, M. D.; Perrin, M. J.; Mann, S. A.; Ke, Y.; Hill, A. P. HERG K + Channels: Structure, Function, and Clinical Significance. Physiological Reviews 2012, 92 (3), 1393–1478. https://doi.org/10.1152/physrev.00036.2011.
(2) Slavov, S.; Zhao, J.; Huang, R.; Xia, M.; Beger, R. Prediction and Experimental Evaluation of the HERG Blocking Potentialof Drugs Showing Clinical Signs of Cardiotoxicity. LDDD 2023, 20 (11), 1757–1767. https://doi.org/10.2174/1570180819666220804110706.
(3) International Conference On Harmonisation Of Technical Requirements For Registration Of Pharmaceuticals For Human Use. In Handbook of Transnational Economic Governance Regimes; Tietje, C., Brouder, A., Eds.; Brill | Nijhoff, 2010; pp 1041–1053. https://doi.org/10.1163/ej.9789004163300.i-1081.897.
(4) Al-Khatib, S. M.; Stevenson, W. G.; Ackerman, M. J.; Bryant, W. J.; Callans, D. J.; Curtis, A. B.; Deal, B. J.; Dickfeld, T.; Field, M. E.; Fonarow, G. C.; Gillis, A. M.; Granger, C. B.; Hammill, S. C.; Hlatky, M. A.; Joglar, J. A.; Kay, G. N.; Matlock, D. D.; Myerburg, R. J.; Page, R. L. 2017 AHA/ACC/HRS Guideline for Management of Patients With Ventricular Arrhythmias and the Prevention of Sudden Cardiac Death: Executive Summary: A Report of the American College of Cardiology/American Heart Association Task Force on Clinical Practice Guidelines and the Heart Rhythm Society. Circulation 2018, 138 (13). https://doi.org/10.1161/CIR.0000000000000548.